Brighter than a billion suns
Scientists at Albert Einstein Institute calculate the first complete relativistic simulation of colliding neutron stars
What happens exactly when two neutron stars collide and form a black hole? How much energy is released in this event? Is this the way in which the enigmatic gamma-ray bursts are produced? The numerical-relativity group at the Max Planck Institute for Gravitational Physics (Albert Einstein Institute, AEI) has now answered these questions and published the first complete and accurate simulations of this complex process in three dimensions and under full conditions of general relativity. The results yield important information both for gravitational wave- and for gamma-ray burst-researchers and hence to a deeper understanding of our universe. The paper was now published in the scientific journal Physical Review D. These calculations, with a complexity that requires the use of the most advanced parallel computers, were performed using the specially dedicated supercomputers at the AEI: BELLADONNA and DAMIANA.
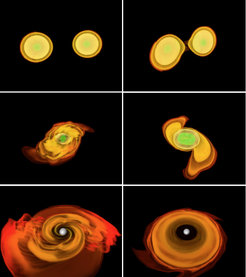
„Our calculations provide the clearest predictions yet of how two neutrons stars merge together.” says Prof Luciano Rezzolla, head of the numerical-relativity group at AEI. “Neutron stars are extremely fascinating objects and, when compared to black holes, their physics is much more interesting and rich. Our calculations of their behaviour provide new information about the gravitational waves emitted, but also about short gamma-ray bursts (or GRBs), some of the most energetic and mysterious astrophysical explosions”
Prof. Bernard Schutz, Managing Director of the AEI adds: “The results obtained by Prof. Rezzolla’s group represent a very important step in understanding collisions of neutron stars. The group has worked for many years to develop the most powerful software tools, and they have been supported by the Max Planck Society with some of the fastest computers available for this kind of research. This long effort has led to today’s breakthrough result. We finally have a simulation that uses all the physics of Einstein’s general relativity to study fully these rare but extremely powerful events.”
Colliding neutron stars in a supercomputer
The AEI simulations are the results of many years of research and code development and represent the state of the art in this research area for several different reasons:
- Firstly, they provide the first complete picture of this process, starting from the inspiral phase (when the stars are far separated and interact only gravitationally) up to their merger and over to the formation of the black hole/torus system. The complete evolution is very clearly described in terms of the gravitational-wave emission and the simulations allow one to distinguish very clearly the slow inspiral phase from the very dynamical stage when a single a hyper-massive neutron star (or HMNS) is formed and also how this star subsequently evolves before collapsing to a black hole.
- Secondly, these simulations are the most accurate to date and have allowed the scientists at the AEI to study not only the bulk motion of the two stars as they inspiral, but also the complex physical processes and instabilities which develop when the two neutron stars merge. Some of these instabilities are very energetic and could lead to very interesting astrophysical observations and, in particular, to the generation of ultra-high magnetic fields which would then serve to launch and collimate energetic jets of plasma. The occurrence of such instability has been conjectured in the past, but the simulations performed at the AEI are the first ones where the instability is seen to develop in self-consistent and general relativistic calculations.
- Finally, the simulations performed at the AEI also have an important impact on the modelling of gamma-ray bursts (GRBs). We know that they are the result of very energetic and catastrophic events, releasing in a few seconds the same amount of energy that the entire Galaxy releases in one year. One of the most widely accepted scenarios behind the formation of a “short” GRB involves the merger of two neutron stars and the formation of a black hole surrounded by a torus. This scenario has been conjectured essentially at the same time as the first detections, but whether or not it could be produced in practice has remained an unsolved issue for many years. The calculations at the AEI have now clearly shown that this scenario does indeed take place and follows naturally from the merger of two neutron stars. While confirming similar previous studies, the simulations at the AEI have provided for the first time the evidence that the torus around the black hole is massive, very hot and, most importantly, stable over many orbital periods. These conditions are essential for the emission of neutrino and anti-neutrino pairs whose annihilation would yield the powerful gamma-ray emission.
Two neutron stars collide and a new black hole forms – the whole process
The AEI simulations consider the stars when they are still widely separated and then evolve them until their orbits progressively shrink as a result of the emission of gravitational waves. Once too close to resist each other’s gravity, the two neutron stars violently merge to produce a hyper-massive neutron star (or HMNS) that eventually collapses to produce a rotating black hole. The latter is surrounded by a torus of hot and dense matter that is spinning too rapidly to fall into the black hole and will orbit around it until it is slowly but progressively accreted.
The whole process of late inspiral, merger and collapse to a black hole is very rapid, and it is also extremely energetic. Indeed, in less than 1 sec, the binary system releases the same amount of energy that our Sun emits in ten billion years; as a result, the binary would be “visible” (to the eyes of powerful detectors) even from a billion light years away.
Even though one of the most widely accepted scenarios behind the formation of a “short” gamma-ray burst involves the merger of two neutron stars and the formation of a black hole surrounded by a torus, the calculations at AEI are the first ones to clearly show that this scenario does indeed take place and is the natural result of the merger of two neutron stars. The simulations also provide convincing evidence that the torus around the black hole is massive, very hot and, most importantly, stable over many orbital periods. As mentioned above, these conditions are essential for the generation of powerful gamma-ray emission.
Neutron stars
Together with black holes, neutron stars are among of the most fascinating objects in the universe. The end-result of stellar evolution and the remnant of a powerful supernova explosion, neutron stars have a mass which is slightly larger than the mass of the Sun (< 1.4M⊙), but which is compressed in almost perfect spheres with a radius not larger than a small town (< 10 − 12 km). As a result, the matter which neutron stars are composed of is extreme in many respects. Their density, for instance, is so high that a single tablespoon of neutron-star matter would weigh as much as the entire Alpine mountain chain. At the same time, the gravitational forces are so strong that the physical conditions are very close to those in the vicinity of a black hole of comparable mass. Clearly, such conditions cannot be reproduced on terrestrial laboratories and because of this, much of these fascinating objects is still unknown.
The internal structure of neutron stars
A great part of our knowledge about the size and mass of neutron stars comes from satellite observations in the X- and gamma-ray bands, and which reveal the activities of these compact stars when they are in binary systems and accreting the matter which they have stripped gravitationally from the massive companion. However, because these observations come from electromagnetic signals, they cannot probe the internal structure of neutron stars but only provide indications on the physical conditions at the stellar surface. When observed as “pulsars” in the radio band, neutron stars reveal that they are often endowed with very large magnetic fields. Unfortunately these observations can probe only the regions of the neutron star lying above its surface and are essentially blind about the stars’ interior.
However, electromagnetic signals are not the only way in which neutrons stars can be observed. Because of the extreme (or relativistic) conditions they experience and produce, neutron stars are in fact also expected to emit large amounts of gravitational radiation, either when oscillating or when orbiting around each other in a binary system that gradually shrinks. Indeed, binary systems of neutron stars are already observed in the radio bands: e.g. PSR 1913+16 which gave the Nobel prize to Russell A. Hulse and Joseph H. Taylor in 1993, or the recently discovered binary PSR J0737-3039A/B. Such binary systems are among the most powerful sources of gravitational waves and could be detected already by present gravitational wave observatories if observed in the final stages of the inspiral and if sufficiently close to us. Although gravitational waves have not yet been revealed experimentally, they do not suffer from the limitations imposed on electromagnetic waves and would provide information not only on the mass and radius of the stars, but also on the interior structure and composition of neutron stars. Once they are detected, the gravitational waves from neutron stars would work as the “Rosetta” stone to decipher the interior of neutrons stars.
Status of current gravitational wave detectors
Currently, several first generation gravitational wave detectors are active in Europe. The German-British GEO600 observatory, operated by the AEI and funded by STFC (Science and Technology Facilities Council) and MPG (Max Planck Gesellschaft), is operating close to Hannover while the French-Italian-Dutch Virgo project is located in Cascina near Pisa (Italy). These interferometers pool their data with the three American LIGO interferometers and are currently doing extensive searches for gravitational waves from astrophysical systems.
During the next decade all interferometric gravitational wave detectors will be upgraded to second- generation instruments. Virgo and LIGO will gain a factor of about ten in sensitivity at lower frequencies (up to about a kilohertz) using technology developed in Europe and elsewhere. GEO will pioneer high frequency wide-band observing above one kilohertz, again deploying new technologies.
Calculating gravitational wave signals from merging neutron stars
Besides the enormous experimental difficulties related with the detection of the gravitational-wave signal from neutron stars (the signals arriving on Earth are in fact extremely small) there is the considerable difficulty of calculating the gravitational-wave signal from such a system. Knowing what the waveforms look like would enhance the detection probability (the experimentalist would know what signal to detect from their noise apparatuses) and also allow the reconstruction of the actual stellar structure. However, the theoretical prediction of these waveforms is made difficult by the fact that it involves the numerical solution of the Einstein equations – a set of nonlinear, coupled differential equations – as well as of the equations of relativistic hydrodynamics (the equations governing the motion of matter). The numerical-relativity group at the AEI has developed, over the last five years, a number of numerical codes aimed at computing such waveforms under a variety of different conditions ranging from binary systems of black holes, over to binary systems of neutron stars.